PERSPECTIVES mammalian nervous system.
Sample Answer for PERSPECTIVES mammalian nervous system. Included After Question
PERSPECTIVES mammalian nervous system.
Question Description
I’m working on a psychology discussion question and need support to help me learn.
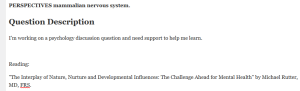
Reading:
“The Interplay of Nature, Nurture and Developmental Influences: The Challenge Ahead for Mental Health” by Michael Rutter, MD, FRS.
Caspi, A., & Moffitt, T. E. (2006). Gene–environment interactions in psychiatry: joining forces with neuroscience. Nature Reviews Neuroscience,7 (7), 583-590.
“Too small to Fail” by Nicholas Kristof, New York Times, June 2, 2016.
Film:
Wonder (d. Steven Chbosky, 2017, USA)
A Sample Answer For the Assignment: PERSPECTIVES mammalian nervous system.
Title: PERSPECTIVES mammalian nervous system.
Curr. Opin. Neurobiol. 14, 617–628 (2004). 104. Miyawaki, A. Innovations in the imaging of brain functions using fluorescent proteins. Neuron 48, 189–199 (2005). 105. Barco, A., Alarcon, J. M. & Kandel, E. R. Expression of constitutively active CREB protein facilitates the late phase of long-term potentiation by enhancing synaptic capture. Cell 108, 689–703 (2002). 106. Woo, N. H. & Nguyen, P. V. ‘Silent’ metaplasticity of the late phase of long-term potentiation requires protein phosphatases. Learn. Mem. 9, 202–213 (2002). 107. Woo, N. H. & Nguyen, P. V. Protein synthesis is required for synaptic immunity to depotentiation. J. Neurosci. 23, 1125–1132 (2003). 108. Fiala, J. C. & Harris, K. M. in Dendrites (eds Stuart, G., Spruston, N. & Häusser, M.) 376 (Oxford Univ. Press, New York, 1999). Acknowledgements We thank C. Stevens, M. Wilson and members of the Tonegawa laboratory for helpful discussions, and critical reading of and comments on the manuscript. Research was supported by the RIKEN-MIT Neuroscience Research Center, Howard Hughes Medical Institute and grants from the National Institutes of Health (S.T. and R.J.K). Competing interests statement The authors declare no competing financial interests. DATABASES The following terms in this article are linked online to: Entrez Gene: http://www.ncbi.nlm.nih.gov/entrez/query. fcgi?db=gene 4EBP1 | 4EBP2 | BDNF | eEF1α | eIF4E | S6 Access to this links box is available online. OPINION Gene–environment interactions in psychiatry: joining forces with neuroscience Avshalom Caspi and Terrie E. Moffitt Abstract | Gene–environment interaction research in psychiatry is new, and is a natural ally of neuroscience. Mental disorders have known environmental causes, but there is heterogeneity in the response to each causal factor, which gene– environment findings attribute to genetic differences at the DNA sequence level. Such findings come from epidemiology, an ideal branch of science for showing that gene–environment interactions exist in nature and affect a significant fraction of disease cases. The complementary discipline of epidemiology, experimental neuroscience, fuels gene–environment hypotheses and investigates underlying neural mechanisms. This article discusses opportunities and challenges in the collaboration between psychiatry, epidemiology and neuroscience in studying gene–environment interactions. Gene–environment interactions occur when the effect of exposure to an environmental pathogen on a person’s health is conditional on his or her genotype. The first evidence that genotype moderates the capacity of an environmental risk to bring about mental disorders was reported in 2002 (REF. 1). Although mental health research into gene–environment interactions is new, it seems to be gathering momentum. We argue that, to fulfill its potential, gene–environment interaction research must integrate with neuroscience. Moreover, the gene–environment interaction approach brings exciting opportunities for extending the range and power of neuroscience. Here, we examine opportunities for collaboration between experimental neuroscience and research on NATURE REVIEWS | NEUROSCIENCE gene–environment interactions. Successful collaboration can solve the biggest mystery of human psychopathology: how does an environmental factor, external to the person, get inside the nervous system and alter its elements to generate the symptoms of a disordered mind? Concentrating the considerable resources of neuroscience and gene–environment research on this question will bring discoveries that advance the understanding of mental disorders, and increase the potential to control and prevent them. Psychiatric genetic approaches The recent history of psychiatric research that has measured genetic differences at the DNA sequence level can be divided into three approaches, each with its own logic and assumptions. The first approach assumes direct linear relations between genes and behaviour (FIG. 1a). The goal of this approach has been to correlate psychiatric disorders with individual differences in DNA sequence. This has been attempted using both linkage analysis and association analysis, with regard to many psychiatric conditions such as depression2, schizophrenia3 and addiction4. Although a few genes have accumulated replicated evidence of association with disorder, replication failures are routine and overall progress has been slow5. Because of inconsistent findings, many scientists have despaired of the search for a straightforward association between genotype and diagnosis6, that is, for direct main effects. The second approach has sought to make more progress by replacing the disorder outcomes with intermediate phenotypes, called ‘endophenotypes’ (FIG. 1b). Endophenotypes are heritable neurophysiological, biochemical, endocrinological, neuroanatomical or neuropsychological constituents of disorders7. Endophenotypes are assumed to have simpler genetic underpinnings than disorders themselves. Therefore, this research approach pursues the hypothesis that it will be easier to identify genes associated with endophenotypes than genes associated with their correlated disorders. Although this approach substitutes the psychiatric diagnosis with an intermediate brain measure, it still searches for direct main effects. The third approach to psychiatric genetics, unlike the first two approaches, seeks to incorporate information about the environment (FIG. 1c). This gene–environment interaction approach differs fundamentally from the ‘main-effect approaches’, with regard to the assumptions about the causes of psychiatric disorders. Maineffect approaches assume that genes cause disorder, an assumption carried forward from early work that identified single-gene causes of rare Mendelian conditions. By contrast, the gene–environment interaction approach assumes that environmental pathogens cause disorder, and that genes influence susceptibility to pathogens. In contrast to main-effect studies, there is no necessary expectation of a direct gene-tobehaviour association in the absence of the environmental pathogen. The gene–environment interaction approach has grown out of two observations: first, that mental disorders have environmental causes; second, that people show heterogeneity in their response to those causes8. VOLUME 7 | JULY 2006 | 583 PERSPECTIVES a Gene b Gene Disorder Endophenotype c Environment Disorder Disorder Genotype d Environment Neural substrate reactivity Disorder Genotype Figure 1 | Approaches to psychiatric genetics research. a | The gene-to-disorder approach assumes direct linear relations between genes and disorder. b | The endophenotype approach replaces the disorder outcomes with intermediate phenotypes. c | The gene–environment interaction approach assumes that genes moderate the effect of environmental pathogens on disorder. d | Neuroscience complements the latter research by specifying the proximal role of nervous system reactivity in the gene–environment interaction. Nature and nurture Like other non-communicable diseases that have common prevalence in the population and complex multi-factorial aetiology, most mental disorders have known non-genetic, environmental risk factors (that is, predictors whose causal status is unproven) and/or environmental pathogens (that is, proven causes)9,10. Environmental pathogens have been documented for substance-use disorders11, antisocial disorders12, depression13, and even schizophrenia-spectrum disorders14,15. The pool of environmental factors is currently more limited for disorders such as autism, Alzheimer’s-type dementia, and attentiondeficit hyperactivity disorder (ADHD). Nevertheless, the concordance of monozygotic twins for even these highly heritable disorders is less than perfect, indicating the existence of non-genetic contributing causes. Environmental risk factors for mental disorders discovered to date include (but are not limited to) maternal stress during pregnancy, maternal substance abuse during pregnancy, low birth weight, birth complications, deprivation of normal parental care during infancy, childhood physical maltreatment, childhood neglect, premature parental loss, exposure to family conflict and violence, stressful life events involving loss or threat, substance abuse, toxic exposures and head injury. These environmental causes are considered to be only contributory because exposure to them does not always generate disorder. Both human and animal studies consistently reveal variability in individuals’ behavioural responses to environmental pathogens. Heterogeneity of response characterizes all known environmental risk factors 584 | JULY 2006 | VOLUME 7 for psychopathology, including even the most overwhelming of traumas. Such response heterogeneity is associated with pre-existing individual differences in temperament, personality, cognition and autonomic physiology, all of which are known to be under genetic influence16. The hypothesis of genetic moderation implies that differences between individuals, originating in the DNA sequence, bring about differences between individuals in their resilience or vulnerability to the environmental causes of many pathological conditions of the mind and body. This pathogenesis hypothesis is under study in relation not only to mental disorders, but also to cancer17, diabetes18, and cardiovascular19, immune/infectious20,21 and respiratory22 diseases. Gene–environment interaction studies in psychiatry are new, but some of the initial findings are intriguing. Our own studies provided proof of principle of this approach. In the first report of gene–environment interaction in relation to behaviour, we tested the hypothesis that a functional polymorphism in the promoter region of the gene encoding the neurotransmitter-metabolizing enzyme monoamine oxidase A (MAOA) would moderate the effect of child maltreatment in the cycle of violence. Results showed that maltreated children, whose genotype conferred low levels of MAOA expression, more often developed conduct disorder, antisocial personality and adult violent crime than children with a high-activity MAOA genotype1. In a second study, we proposed that a functional polymorphism in the promoter region of the serotonin transporter (5-HTT) gene would moderate the influence of stressful life events on depression. Individuals with one or two copies of the 5-HTT ‘short’ allele exhibited more depressive symptoms, diagnosable depression, and suicidality following stressful life events than individuals with two copies of the ‘long’ allele23. A third study, by investigating the differential effects of cannabis on its users, demonstrated that gene–environment interactions involve environmental pathogens apart from psycho-social risks. We suggested that a functional polymorphism in the catechol-O-methyltransferase (COMT) gene would moderate the link between adolescent cannabis use and risk of developing adult psychosis. Cannabis users carrying the COMT valine allele were likely to exhibit psychotic symptoms and to develop schizophrenia-spectrum disorder, but cannabis use had no such adverse influence on individuals with two copies of the COMT methionine allele24. Additional gene–environment findings are emerging. In two studies of ADHD, polymorphisms in the dopamine system interacted with antenatal risk factors (for example, low birth weight and maternal use of alcohol) to predict key symptoms associated with the disorder25,26. In another report, polymorphisms in the glucocorticoid receptor-regulating gene FKBP5 interacted with acute injury to predict psychological dissociation, a key feature of post-traumatic stress syndrome27. The study of gene–environment interactions has been the province of epidemiology, in which genotypes, environmental pathogen exposures and disorder outcomes are studied as they naturally occur in the human population28. Genetic epidemiology is ideal for achieving three goals. First, epidemiological studies identify the involvement of hypothesized gene–environment interactions. Second, to increase confidence in the interaction, epidemiological studies incorporate control factors necessary for ruling out alternative explanations. Third, epidemiological studies attest whether an interaction accounts for a non-trivial proportion of the disorder in the human population. However, genetic epidemiology is limited for understanding the biological mechanisms involved in an interaction, and therefore its potential will be better realized when it is integrated with experimental neuroscience. Neuroscience can complement psychiatric genetic epidemiology by specifying the more proximal role of nervous system reactivity in the gene–environment interaction (FIG. 1d). Such information about proximal mechanisms will be essential for developing theory and treatments. www.nature.com/reviews/neuro PERSPECTIVES ‘Bootstrapping’ with neuroscience The original impetus for conducting each of our epidemiological gene–environment interaction studies came from findings that had been established by neuroscience research. We have subsequently observed that, once a novel gene–environment interaction is reported, a wave of new neuroscience follows. This suggests a mutually beneficial relationship of ‘bootstrapping’ between the two fields (FIG. 2). In the initial phase of research into gene– environment interactions, neuroscience provides building blocks that are needed to construct a hypothesis (FIG. 2a). The building blocks correspond to the three elements of the triad: the disorder, the environmental pathogen and the genotype. First, evidence is needed about which neural substrate is involved in the disorder. Second, evidence is needed that an environmental cause of the disorder has effects on variables indexing the same neural substrate. Third, evidence is needed that a candidate gene has functional effects on variables indexing that same neural substrate. It is this convergence of environmental and genotypic effects within the same neural substrate that allows for the possibility of gene–environment interactions. At present, such evidence concerning environmental and genotypic effects in relation to neural substrate measures is sparse, and therefore gene–environment interaction hypotheses are likely to be circumstantial at best, and flimsy at worst. But this situation is steadily improving. When we were constructing our hypothesis regarding the genetic moderation of the depressogenic effects of stressful life events23, we were aided by direct evidence linking the 5-HTT candidate gene to individual differences in physiological responsiveness to stress conditions in three different experimental paradigms, including knockout mice29, stress-reared rhesus macaques30 and human functional brain imaging31. Such helpful studies are uncommon as yet, but they are emerging. In the second (epidemiological) phase of research, the new gene–environment interaction hypothesis is tested against data (FIG. 2b). Elsewhere, we have discussed potential pitfalls of gene–environment interaction studies and have outlined strategies to guide this research8,32. If the initial data are consistent with the hypothesis, the finding must be replicated to determine whether it is sufficiently reliable to warrant further neuroscience investigations33. Most gene–environment interaction findings have emerged too recently to be evaluated according to their replication records. NATURE REVIEWS | NEUROSCIENCE a Neuroscience evidence base b Epidemiological gene–environment interaction research c Experimental neuroscience Neuroscience Gene–envionment interaction Neuroscience Building blocks Hypothesis New studies D 1 Disorder links to neural substrate N 2 Environment affects neural substrate N 3 Genotype affects neural substrate N Finding Genetic variation in neurosystem responses to environments N G×E→D G E Figure 2 | Integrating neuroscience and gene–environment interaction research. Neuroscience provides the building blocks for constructing hypotheses about gene–environment interaction (a) that are tested against data (b), subsequently stimulating new studies to illuminate the black box of biology (c) between the gene (G), the environmental pathogen (E) and the disorder (D). However, two of these findings are promising. First, several studies have sought to replicate the interaction between the highand low-activity MAOA genotypes and maltreatment34–38; a meta-analysis revealed a significant pooled effect36. Second, positive replications of the interaction between 5-HTT*long/5HTT*short genotypes and life stress have also appeared39–47, along with two failures to replicate48,49. It is important to note that useful information can also be gleaned from inconsistencies across study findings. For example, as more studies accumulate it will be possible to evaluate whether the moderating effect of the 5-HTT genotype on life stress is stronger among females or males, younger adults or older adults, and first-onset or recurrent depression cases. In the third phase of research, scientific activity comes a full circle, back to neuroscience (FIG. 2c). A new wave of studies is stimulated, each aiming to illuminate the black box of biology between the gene, the environmental pathogen, and the disorder50 (as illustrated in the triangle in FIG. 2). For example, evidence that variation in the promoter region of the 5-HTT gene shapes depressogenic responses to life stress has led to more focused neuroscience research on a genetic susceptibility mechanism for stressrelated depression51–54. Similarly, evidence that a polymorphism in the MAOA gene might contribute to the cycle of violence in maltreated children1 — a hypothesis stimulated by behavioural evidence from mouse knockouts for MAOA55 and functional gene knockouts in humans56 — has, in turn, stimulated efforts to probe circuits of emotional arousal in the brain by studying this polymorphism in imaging paradigms57 (see also BOX 1). Enhancing neuroscience A replicated finding on gene–environment interactions adds new information, producing a stimulating effect on neuroscience. The result of a reliable gene–environment interaction finding is clear evidence for a pathway of causal neural process connecting the three disparate ‘end points’ that form the triad of gene, environmental pathogen and disorder. The pathway might initially be hidden from scientific view, but knowing three endpoints (instead of two) enhances the likelihood of finding the neurobiological paths that unite them. Candidate genes can add information about where in the body, cell and molecule the environmental pathogen’s effect on disorder occurs. A replicated finding on gene–environment interactions yields at least three insights. First, the insight that the result of exposure to an environmental pathogen depends on the person’s genotype offers clues about the root beginnings of a causal pathway. Variation in the DNA sequence antedates all other variables in the triad. Therefore, covariation between a measured genotype and a neural substrate variable is useful for making deductions about the position of the neural substrate variable in the causal chain. For example, if a study showed that amygdala activation in response to emotional stimuli was abnormal in depressed subjects, this could indicate either VOLUME 7 | JULY 2006 | 585 PERSPECTIVES Box 1 | How does genotype moderate the psychological effects of cannabis use? Evidence from studies around the world shows that cannabis use is a statistical risk factor for the emergence of psychosis, ranging from psychotic symptoms (such as hallucinations and delusions) to clinically significant disorders (such as schizophrenia)93. However, most people who use cannabis do not develop psychosis, which suggests that some individuals may be genetically vulnerable to its effects. This hypothesis received initial support from research showing that the association between cannabis use and psychosis outcome is most marked in subjects with an established vulnerability to psychosis94. However, the genetic risk involved was not specified. Subsequent research focused on risk measured by individual differences on the catechol-O-methyltransferase (COMT) gene; in particular, a valine allele at codon 158 producing more enzymatic activity and faster breakdown of dopamine than the methionine allele. Both the COMT valine allele77 and cannabis use95 have been independently associated with brain endophenotypes for schizophrenia96,97. An epidemiological study (see panel a) that traced a longitudinal cohort from prior to the onset of cannabis use (age 11 years), through to the peak risk period of psychosis onset (age 26 years), revealed that individuals with one or more high-activity valine alleles (VAL/METor VAL/VAL) showed subsequent increased risk of psychotic symptoms and psychosis-spectrum disorder if they used cannabis24. Cannabis use had no such adverse influence on individuals with two copies of the methionine allele (MET/MET). But is the quantification of drug exposure information using the self-reports of adolescent subjects sufficiently accurate? Is it possible that valine-allele carriers who use cannabis are unusual in some unmeasured way? And how does the valine allele influence sensitivity to cannabis? These questions have been addressed by researchers in the Netherlands, who used an experimental design to extend the epidemiological finding98. In their studies, subjects were tested on two occasions, separated by 1 week, as part of a double-blind, placebo controlled cross-over design. In randomized order, they received either 0 µg or 300 µg -9-tetrahydrocannabinol (the principal component of cannabis) per kilogram bodyweight. Cannabis affected cognition and state psychosis, but this was conditional on COMT genotype. As illustrated in panel b, individuals carrying two copies of the valine allele exhibited more cannabis-induced memory and attention impairments than carriers of the methionine allele, and were the most sensitive to cannabis-induced psychotic experiences. Further research — including the use of both animal and imaging paradigms — is needed to provide a fuller understanding of genetically moderated responses to cannabis99. a b 12 Memory performance: delayed recognition scores on a visual memory task 15 10 5 10 VAL/MET VAL/VAL COMT genotype No adolescent cannabis use Adolescent cannabis use a causal role for the amygdala in depression, or a consequence of depression on the amygdala. However, if such amygdala activation depends on the subjects’ genotype, this suggests that amygdala activation has precedence. Such precedence is not sufficient for causation, but it is necessary. Second, awareness of gene–environment interactions can help to reveal stronger effects 586 | JULY 2006 | VOLUME 7 MET/MET VAL/MET 0) 0) (2 (2 5) 5) (3 (3 (1 9) = 9) n 48 ) (5 4) (1 (3 (1 MET/MET 11 ) (9 1) 9 n = 51 ) (4 8) 0 11 (1 Schizophreniform disorder in adulthood (%) 20 VAL/VAL COMT genotype Placebo condition Cannabis treatment condition in neuroscience data. Neuroscience variables are generally responsive to environmental input. If responsiveness is under the influence of hidden genetic variation within a research sample, this unmeasured heterogeneity will dilute findings. Returning to the prior example, amygdala activation to an emotional stimulus can appear positive but weak across all subjects in an experiment, as the result of unwittingly averaging data from two genotype groups, one of strong responders and another of non-responders. If genetically vulnerable subgroups can be identified for analysis, modest associations may be revealed as stronger than previously thought. Third, gene–environment interactions might help to solve the perennial riddle of disorder-specific pathophysiology. Most environmental pathogens constitute a nonspecific risk for many disorders. For example, smoking influences cancer, osteoporosis, lung disease, heart disease and fetal growth; child maltreatment influences both aggression and depression; birth complications influence both ADHD and schizophrenia. A potential explanation for why there are different outcomes from one environmental pathogen is that the pathogen is connected to each disorder through a different pathophysiological pathway; there is little research into this, although genes of known functionality may offer clues. Furthering gene–environment research Psychiatric genetics has earned an ignoble reputation for its methodological problems, but this reputation should not discourage neuroscientists from bringing genetics into their laboratories to study the genetic moderation of environmental pathogens’ effects on neural substrates. Many initial reports of gene-to-disorder associations proved to be false positives5, prompting the publication of methodological warnings58–60. However, most of the methodological problems arise from the fact that genetic epidemiology is an observational discipline that measures genotypes, environmental risk conditions and disorder outcomes as they naturally occur. This observational method involves several compromises to validity, but the same problems do not afflict the experimental method. Therefore, experimental neuroscience paradigms will benefit gene–environment interaction research by addressing some of the methodological concerns that are now plaguing genetic epidemiology, as explained below. First, there is concern about the need for very large samples in genetics research61. In case-control studies, large samples are needed because genetic effects are expected to be very small. In cohort studies, small effects are also a concern, and there is the added need for large samples due to the fact that the environmental exposure and/or the disorder might have a low prevalence in cohorts33. By contrast, experimental studies have more control over the group sizes and intensity of environmental stimulus needed www.nature.com/reviews/neuro PERSPECTIVES to obtain a detectable effect62. Moreover, unlike mental disorders, neural substrate outcome measures (such as emotional arousal or adrenocorticotropic hormone responses) tend to be quantitatively distributed such that low prevalence is not at issue. Second, there is concern about gene– environment correlation63,64. When genes influence the probability of subjects’ exposure to an environmental pathogen, this results in the contamination of measures of environmental exposure with genetic variation, thereby clouding interpretation of the findings. For example, the probability of experiencing certain stressful life events is known to be under partial genetic influence, as is the tendency to expose oneself to environmental pathogens such as cannabis or tobacco. By contrast, experimental random assignment of subjects to the environmental risk condition rules out this type of self-selection. For example, epidemiologists study self-initiated cigarette smoking, while neuroscientists can study participants that are randomly assigned to nicotine exposure. Third, there is concern about the difficulty of achieving precise and reliable measures of environmental exposure, particularly if the exposure typically occurs over extended periods of the life course8,65. For example, it is very difficult to ascertain the frequency, timing and extent of the trauma that is entailed in stressful life events. Likewise, it is notoriously difficult, using survey methods, to measure the amount of active drug that is ingested during recreational cannabis use over many years. Experimental administration of the environmental pathogen or stimulus with standardized dosage and timing rules out this concern. Fourth, there is concern about the low prior probability of a true association between a disorder and any one among many thousands of genetic polymorphisms66. If little or nothing is known prior to a statistical test of association between a gene and behaviour, then this results in a low prior probability of the hoped-for association, and any association uncovered could easily be a chance false positive result. Neuroscience research enhances the prior probability of a candidate gene being associated with disorder by connecting that genotype with brain responsiveness to a known environmental cause of the disorder. Thus, a key contribution from experimental neuroscience is evidence and theory that supports the biological plausibility of genetic hypotheses, which helps to prevent false positives. Consider research in cognate medical fields, where caffeine consumption has been linked to the risk of myocardial NATURE REVIEWS | NEUROSCIENCE Exposure to environmental pathogen Infant rearing condition of rhesus macaques Genotype rh-5HTTLPR Mother-reared Short/long Neural substrate reactivity measure ACTH release under stress Pre-stressor Post-stressor 0 100 200 300 400 500 ACTH Long/long Pre-stressor Post-stressor 0 100 200 300 400 500 ACTH Peer-reared Short/long Pre-stressor Post-stressor 0 100 200 300 400 500 ACTH Long/long Pre-stressor Post-stressor 0 100 200 300 400 500 ACTH Figure 3 | Exposure to adverse rearing, genotype and adrenocorticotropin hormone (ACTH) levels. Influence of exposure to early stress (peer rearing) on subsequent exaggerated responses of the limbic-hypothalamic-pituitary-adrenal axis (LHPA) responses to stress is conditioned by serotonin transporter gene promoter variation (rh-5HTTLPR) in rhesus macaques. When exposed to stress later in life, peer-reared animals with the short/long genotype had higher ACTH levels than animals with the long/long genotype. There were no differences between genotypes among animals reared with their mothers (data from REF. 105). infarction. Caffeine is metabolized by an enzyme (CYP1A2) in the liver, knowledge that allowed researchers to test (and confirm) the hypothesis that carriers of the slow metabolizer variant of the CYP1A2 gene are at a heightened risk of myocardial infarction67. As researchers learn more about genes, the brain and environmental pathogens, the prior probability of hypotheses will become stronger, and false positive gene findings fewer. One caveat must be mentioned. Experiments that randomly assign subjects to environmental pathogens will inevitably be limited to using substitutes analogous to the environmental pathogens that cause mental disorders. Real environmental pathogens are not amenable to experimental administration for three reasons: first, ethics prohibit exposing humans to risk; second, animal-model exposures cannot be equated with human exposures; and third, harm from naturally occurring environmental pathogens often accumulates for months or years longer than a laboratory experiment. These shortcomings of experimental gene– environment interaction studies must be acknowledged. However, the shortcomings are diminished where a chain of inference can link experimental findings involving an analogue pathogen to epidemiological findings involving its counterpart natural environmental pathogen. Towards a nomological network A nomological network refers to the interlocking system of laws — the predicted pattern of theoretical relationships — which define a construct68. A chain of inferences is required to validate the claim that specific gene–environment interactions are surrounded by a nomological network of individual supporting findings. In mental health research, such an emerging nomological network is illustrated by many approaches that are used to understand the role of 5-HTT gene variation in emotion regulation and emotional disorders69,70. We hope that the present article will encourage further collaboration between genetic epidemiology and experimental neuroscience in a joint effort to unravel the complex mechanisms that underlie gene–environment interactions. We envisage six ways forward. First, animal models of environmental pathogen exposure are needed (FIG. 3). In non-human animals, both genotype and exposure to a pathogen can be manipulated under experimental control71,72. Studying non-human subjects is an advantage because they can be assigned to detrimental conditions that are not permitted in human studies (for example, deprivation of maternal rearing). These experiments use different strains, genetically modified animals or animals that have known human-relevant VOLUME 7 | JULY 2006 | 587 PERSPECTIVES Box 2 | Bringing genetics into experimental psychopathology The use of experimental models in behavioural genomics is exemplified by research on substanceuse disorders. Rather than search for direct main-effect associations between candidate genes and addiction, this research uses experimental paradigms to identify how genotype moderates subjects’ reactions to environmental stimuli (such as to priming doses or drug cues) that are associated with addictive substances. In one experiment, the researchers investigated whether a functional variable number of tandem repeats (VNTR) polymorphism in the D4 dopamine receptor gene (DRD4) affected craving after priming doses and drug cues. Participants were tested on two occasions, randomly assigned to receive three alcoholic drinks on the first session and three control drinks on the second session, or the reverse. Individuals carrying the DRD4 long (L) allele reported a stronger urge to drink in the alcohol condition than in the placebo condition. By contrast, individuals with two short DRD4 alleles (S) reported no differences in the urge to drink between the two conditions100. Next, the investigators manipulated the putative pharmacological mechanism that mediates the effect of DRD4 on craving. It was suggested that alcohol increases craving through activation at the D4 receptor and that carriers of the DRD4*L allele are especially vulnerable to this effect. Subjects classified as DRD4*L or DRD4*S were administered olanzapine (a D4 antagonist that was proposed to block the ability of alcohol to trigger craving) or cyprohyptadine (a control medication) prior to the alcohol-challenge study. Olanzapine was more effective for DRD4*L subjects, helping to narrow the mediating mechanism involved in genetic control of sensitivity to the environment101,102. These findings suggest that the DRD4 polymorphism moderates craving after alcohol consumption, and indicate that DRD4*L individuals may be more susceptible to losing control over drinking. But the DRD4 polymorphism is not simply a genetic risk for alcohol abuse. Individuals carrying the L allele also experience more craving and arousal after exposure to tobacco smoking cues, whereas DRD4*S individuals do not (data for the panel are from REF. 103). This suggests that DRD4 may influence the incentive salience of appetitive stimuli more generally, and offers a clue as to why different addictive disorders tend to co-occur in the same individuals104. Genotype DRD4 VNTR in tobacco smokers Exposure to environmental stimulus a lit cigarette DRD4 short Neural substrate reactivity measure self-reported craving Baseline Post-exposure 50 60 70 80 90 100 90 100 Craving DRD4 long Baseline Post-exposure 50 60 70 80 Craving polymorphisms. The experiments measure responsiveness through various physiological and behavioural phenotypes. We emphasize the value of animal models of environmental pathogen reactivity, rather than animal models of mental disorders. Animal models of mental disorders have been criticized because they cannot represent core cognitive symptoms of human mental disorders73. By contrast, animal models of genetic susceptibility to environmental pathogens offer a valuable window for understanding the effects of pathogen exposure on disease processes74–76. Second, studies that compare human genotype groups on their responses to experimentally administered environmental stimuli are needed. In the vanguard of such research is imaging genomics, which compares the responses of genotype groups using functional neuroimaging measures77–80. There is untapped potential in other 588 | JULY 2006 | VOLUME 7 experimental psychopathology paradigms. We look towards a new wave of investigations asking whether genotype influences humans’ responsiveness to emotion-eliciting stimuli, laboratory stress paradigms or other analogue environmental pathogens. These human gene–environment experiments will use neurophysiological, biochemical, endocrinological, neuroanatomical, cognitive, emotional or neuropsychological measures as phenotypes. Likely examples might include peripheral psychophysiological measures such as the electroencephalogram, electrodermal or heart rate reactivity81–83 and adreno-cortical reactivity84 (see also BOX 2). Third, more epidemiological cohort studies should collect neuroscience measurements. Many ongoing cohort studies are now adding DNA to their data collection protocols. These longstanding cohort studies already have prospective longitudinal histories of participants’ environ- mental exposures and mental disorders that make them ideal for gene–environment interaction research, if their participants’ genotypes are characterized8. New cohort studies of gene–environment interactions are also being planned85,86. To the extent that these studies incorporate neuroscience measures of individual differences (for example, neuropsychological tests, heart rate reactivity and immune-system markers), they will create opportunities to integrate experimental and epidemiological findings. Taking neuroscience measurements in large cohorts can be costly and, for functional imaging paradigms, prohibitive. However, with more measures in common, epidemiological findings about genetically moderated environment-to-disorder associations can be integrated with experimental findings about genetically moderated environment-to-brain associations (FIG. 1d). Fourth, the characterization of subjects’ genetic vulnerability as opposed to their resilience needs to move beyond single genetic polymorphisms. New approaches will use information about biological pathways to identify gene systems and study sets of genetic polymorphisms that are active in the pathophysiology of a disorder87. For example, in relation to depression, information about the biology of psycho-social stress88–90 can be used as a first step to characterize a set of genes that define a genotype that is vulnerable as opposed to resilient to stressful life events. Incorporating information about genetic pathways into gene–environment interaction studies will enhance explanatory power, but it will also present unique statistical challenges related to the use of data-mining tools and the pooling of data across different studies33. Fifth, although we have largely focused on testing hypotheses about gene–environment interactions using candidate genes, the gene–environment interaction approach might also aid the identification of new genes that are responsible for vulnerability to a particular disease. Genomewide scans for new disease genes, like most designs in psychiatric genetics, aim to discover genes that have direct main effects on disease susceptibility91. However, this maineffects approach is inefficient for detecting new genes whose effects are conditional on environmental risk. As a result, genes that show no direct connection to disorders in genome-wide scans may nevertheless be connected to disorder through hidden gene–environment interactions. Genomewide scans might be more powerful if ‘gene hunters’ recruit samples selected for known exposure to an environmental pathogen for www.nature.com/reviews/neuro PERSPECTIVES the disorder they wish to study, and then scan for genetic variants in subjects who have, versus those who have not, developed the disorder8. Known environmental pathogens might be profitably exploited as research tools for gene hunting. Sixth, any serious initiative to understand aetiology and inform prevention, including genetics, must be able to explain fundamental demographic patterns of disorder. The most solid facts we have about most mental disorders are that prevalence and incidence vary according to age and sex. There are two leading contenders for explaining these differences92. First, the demographic groups (such as males and females) could be equally vulnerable to causal factors, but differentially exposed to them. Alternatively, the demographic groups could be equally exposed to causal factors, but differentially vulnerable to them. To date, lacking a good empirical handle on biological vulnerability, research has made little progress towards understanding age and sex differences in mental disorders. Gene–environment interaction research, with its focus on hypotheses of environmental exposure and biological vulnerability, is ideally suited to investigate age and sex differences. Mental disorders have well-documented environmental causes. But why do some people who are exposed to an environmental pathogen develop mental disorders, while others do not? Why do some disorders excessively afflict one sex or one age group? How can two people experiencing the same environmental pathogen later develop very different disorders? How does an environmental pathogen, especially one that is psycho-social in its nature, get under the skin to alter the nervous system and generate mental disorders? All of these important questions are questions about the interaction between diathesis and stress, between host and pathogen and, in essence, between genotype and environment. Neuroscience and gene–environment interaction research are joining forces to look for answers. Avshalom Caspi and Terrie Moffitt are at the Medical Research Council Social, Genetic and Developmental Psychiatry Centre, Institute of Psychiatry, King’s College London, P0 Box 80 De Crespigny Park, London SE5 8AF, UK. They are also at the Department of Psychology, University of Wisconsin, 1202 West Johnson Street, Madison, Wisconsin 53706, USA. Correspondence to A.C. or T.E.M. e-mails: a.caspi@iop.kcl.ac.uk; t.moffitt@iop.kcl.ac.uk. doi:10.1038/nrn1925 NATURE REVIEWS | NEUROSCIENCE 1. 2. 3. 4. 5. 6. 7. 8. 9. 10. 11. 12. 13. 14. 15. 16. 17. 18. 19. 20. 21. 22. 23. 24. 25. 26. 27. Caspi, A. et al. Role of genotype in the cycle of violence in maltreated children. Science 297, 851–854 (2002). Levinson, D. F. The genetics of depression. Biol. Psychiatry 18 Nov 2005 (doi: 10.1016/ j.biopsych.2005.08.024). Owen, M. J., Williams, N. H. & O’Donovan, M. C. The molecular genetics of schizophrenia: new findings promise new insights. Mol. Psychiatry 9, 14–27 (2004). Goldman, D., Orozsi, G. & Ducci, F. The genetics of addictions: uncovering the genes. Nature Rev. Genet. 6, 521–532 (2005). Insel, T. & Collins, F. S. Psychiatry in the genomics era. Am. J. Psychiatry 160, 616–620 (2003). Hamer, D. Rethinking behavior genetics. Science 298, 71–72 (2002). Gottesman, I. I. & Gould, T. D. The endophenotype concept in psychiatry: etymology and strategic intentions. Am. J. Psychiatry 160, 636–645 (2003). Moffitt, T. E., Caspi, A. & Rutter, M. Strategy for investigating interactions between measured genes and measured environments. Arch. Gen. Psychiatry 62, 473–481 (2005). Moffitt, T. E. The new look of behavioral genetics in developmental psychopathology. Psychol. Bull. 131, 533–554 (2005). Rutter, M. Environmentally mediated risks for psychopathology: research strategies and findings. J. Am. Acad. Child. Adolesc. Psychiatry 44, 3–18 (2005). Heath, A. C. & Nelson, E. C. Effects of the interaction between genotype and environment: research into the genetic epidemiology of alcohol dependence. Alcohol Res. Health 26, 193–201 (2002). Loeber, R. & Farrington, D. P. Serious and Violent Juvenile Offenders: Risk Factors and Successful Interventions (Sage, Thousand Oaks, California, 1998). Kendler, K. S., Gardner, C. O. & Prescott, C. A. Toward a comprehensive developmental model for major depression in women. Am. J. Psychiatry 159, 1133–1145 (2002). Tsuang, M. T., Stone, W. S. & Faraone, S. V. Genes, environment and schizophrenia. Br. J. Psychiatry 178, S18–S24 (2001). van Os, J., Krabbendam, L., Myin-Gerneys, L. & Delespaul, P. The schizophrenia environment. Curr. Opin. Psychiatry 18, 141–145 (2005). Plomin, R., DeFries, J. C., McClearn, G. E. & McGuffin, P. Behavioral Genetics (W. H. Freeman, New York, 2001). King, M.-C., Marks, J. H. & Mandell, J. B. Breast and ovarian cancer risks due to inherited mutations in BRCA1 and BRCA2. Science 302, 643–646 (2003). O’Rahilly, S., Barroso, I. & Wareham, N. J. Genetic factors in type 2 diabetes: the end of the beginning? Science 307, 370–373 (2005). Corella, D. & Ordovas, J. M. Single nucleotide polymorphisms that influence lipid metabolism: interaction with dietry factors. Annu. Rev. Nutr. 25, 341–390 (2005). Smith, M. W. et al. Contrasting genetic influence of CCR2 and CCR5 variants on HIV-1 infection and disease progression. Science 277, 959–965 (1997). Kotb, M. et al. An immunogenetic and molecular basis for differences in outcomes of invasive group A streptococcal infections. Nature Med. 8, 1398–1404 (2002). Kleeberger, S. R. & Peden, D. Gene-environment interactions in asthma and other respiratory diseases. Annu. Rev. Med. 56, 383–400 (2005). Caspi, A. et al. Influence of life stress on depression: moderation by a polymorphism in the 5-HTT gene. Science 301, 386–899 (2003). Caspi, A. et al. Moderation of the effect of adolescentonset cannabis use on adult psychosis by a functional polymorphism in the COMT gene: longitudinal evidence of a gene X environment interaction. Biol. Psychiatry 57, 1117–1127 (2005). Brookes, K.-J. et al. A common haplotype of the dopamine transporter gene associated with attentiondeficit/hyperactivity disorder and interacting with maternal use of alcohol during pregnancy. Arch. Gen. Psychiatry 63, 74–81 (2005). Thapar, A. et al. Catechol-O-methyltransferase gene variant and birth weight predict early-onset antisocial behavior in children with attention-deficit/hyperactivity disorder. Arch. Gen. Psychiatry. 62, 1275–1278 (2005). Koenen, K. C. et al. Polymorphisms in FKBP5 are associated with peri-traumatic dissociation in 28. 29. 30. 31. 32. 33. 34. 35. 36. 37. 38. 39. 40. 41. 42. 43. 44. 45. 46. 47. 48. 49. 50. 51. medically injured children. Mol. Psychiatry 10, 1058–1059 (2005). Khouri, M. J., Millikan, R., Little, J. & Gwinn, M. The emergence of epidemiology in the genomics age. Int. J. Epidemiol. 33, 936–944 (2004). Murphy, D. L. et al. Genetic perspectives on the serotonin transporter. Brain. Res. Bull. 56, 487–494 (2001). Bennett, A. J. et al. Early experience and serotonin transporter gene variation interact to influence primate CNS function. Mol. Psychiatry 7, 188–122 (2002). Hariri, A. R. et al. Serotonin transporter genetic variation and the response of the human amygdala. Science 297, 400–404 (2002). Moffitt, T. E., Caspi, A. & Rutter, M. Measured geneenvironment interactions in psychopathology: concepts, research strategies and implications for research, intervention and public understanding of genetics. Persp. Psychol. Sci. 1, 5–27 (2006). Hunter, D. J. Gene-environment interactions in human diseases. Nature Rev. Genet. 6, 287–298 (2005). Foley, D. L. et al. Childhood adversity, monoamine oxidase A genotype, and risk for conduct disorder. Arch. Gen. Psychiatry 61, 738–744 (2004). Haberstick, B. C. et al. Monoamine oxidase A (MAOA) and antisocial behaviors in the presence of childhood and adolescent maltreatment. Am. J. Med. Genet 135B, 59–64 (2005). Kim-Cohen, J. et al. MAOA, early adversity and geneenvironment interaction predicting children’s mental health: new evidence and a meta-analysis. Mol. Psychiatry (in the press). Nilsson, K. W. et al. Role of monoamine oxidase A genotype and psychosocial factors in male adolescent criminal activity. Biol. Psychiatry 59, 121–127 (2005). Young, S. E. et al. Interaction between MAO-A genotype and maltreatment in the risk for conduct disorder: failure to confirm in adolescent patients. Am. J. Psychiatry 163,1019–1025 (2006). Eley, T. C. et al. Gene-environment interaction analysis of serotonin system markers with adolescent depression. Mol. Psychiatry 9, 908–915 (2004). Grabe, H. J. et al. Mental and physical distress is modulated by a polymorphism in the 5-HT transporter gene interacting with social stressors and chronic disease burden. Mol. Psychiatry 10, 220–224 (2005). Jacobs, N. et al. Stress-related negative affectivity and genetically reduced 5-HTT function: evidence for synergism in shaping risk for depression. Arch. Gen. Psychiatry (in the press). Kaufman, J. et al. Social support and serotonin transporter gene moderate depression in maltreated children. Proc. Natl Acad. Sci. 101, 17316–17321 (2004). Kaufman, J. et al. BDNF-5HTTLPR gene interactions and environmental modifiers of depression in children. Biol. Psychiatry 59, 673–680 (2006). Kendler, K. S., Kuhn, J. W., Vittum, J., Prescott, C. A. & Riley, B. The interaction of stressful life events and a serotonin transporter polymorphism in the prediction of episodes of major depression: a replication. Arch. Gen. Psychiatry 62, 529–535 (2005). Wilhelm, K. A. et al. Life events, first depression onset and the serotonin transporter gene. Br. J. Psychiatry 188, 210–215 (2006). Zalsman, G. et al. A triallelic serotonin transporter gene promoter polymorphism. Am. J. Psychiatry (in the press). Mandelli, L. et al. Interaction between serotonin transporter gene, catechol-O-methyltransferase gene and stressful like events in mood disorders. Int. J. Neuropsychopharmacol. 7 Jun 2006 (doi:10.10 17/51461145706006882). Gillespie, N. A., Whitfield, J. B., Williams, B., Heath, A. C. & Martin, N. G. The relationship between stressful life events, the serotonin transporter (5-HTTLPR) genotype and major depression. Psychol. Med. 35, 101–111 (2005). Surtees, P. G. et al. Social adversity, the serotonin transporter (5HTTLPR) polymorphism and major depressive disorder. Biol. Psychiatry 59, 224–229 (2006). Merikangas, K. & Risch, N. Will the genomics revolution revolutionise psychiatry? Am. J. Psychiatry 160, 625–635 (2003). Ansorge, M. S., Zhou, M., Lira, A., Hen, R. & Gingrich, J. A. Early-life blockade of the 5-HT transporter alters emotional behavior in adult mice. Science 306, 879–881 (2004). VOLUME 7 | JULY 2006 | 589 PERSPECTIVES 52. Fox, N. et al. Evidence for a gene-environment interaction in predicting behavioral inhibition in middle childhood. Psychol. Sci. 16, 921–926 (2005). 53. Hariri, A. R. et al. A susceptibility gene for affective disorders and the response of the human amygdala. Arch. Gen. Psychiatry 62, 146–152 (2005). 54. Pezawas, L. et al. 5-HTTLPR polymorphism impacts human cingulate-amydala interactions: A genetic susceptibility mechanism for depression. Nature Neurosci. 8, 828–834 (2005). 55. Cases, O. et al. Aggressive behavior and altered amounts of brain serotonin and norepinephrine in mice lacking MAOA. Science 268, 1763–1766 (1995). 56. Brunner, H. G., Nelen, M., Breakefield, X. O., Ropers, H. H. & Vanoost, B. A. Abnormal-behavior associated with a point mutation in the structural gene for monoamine oxidase-A. Science 262, 578–580 (1993). 57. Meyer-Lindenberg, A. et al. Neural mechanisms of genetic risk for impulsivity and violence in humans. Proc. Natl Acad. Sci. 103, 6269–6274 (2006). 58. Bearden, C., Reus, V. I. & Freimer, N. B. Why genetic investigation of psychiatric disorders is so difficult. Curr. Opin. Genet. Dev. 14, 280–286 (2004). 59. Colhoun, H. M., McKeigue, P. M. & Smith, G. D. Problems of reporting genetic associations with complex outcomes. Lancet 361, 865–872 (2003). 60. Lohmueller, K. E., Pearce, C. L., Pike, M., Lander, E. S. & Hirschhorn, J. N. Meta-analysis of genetic association studies supports a contribution of common variants to susceptibility to common disease. Nature Genet. 33, 177–182 (2003). 61. Plomin, R. & Crabbe, J. DNA. Psychol. Bull. 126, 806–828 (2000). 62. McClelland, G. H. & Judd, C. M. Statistical difficulties of detecting interactions and moderator effects. Psychol. Bull. 114, 376–390 (1993). 63. Plomin, R. & Bergeman, C. S. The nature of nurture: genetic influence on ‘environmental’ measures. Behav. Brain. Sci. 14, 373–386 (1991). 64. Rutter, M., Moffitt, T. E. & Caspi, A. Gene-environment interplay and psychopathology: multiple varieties but real effects. J. Child. Psychol. Psychiatry 47, 226–261 (2006). 65. Wong, M. Y., Day, N. E., Luan, J. A., Chan, K. P. & Wareham, N. J. The detection of gene-environment interaction for continuous traits: should we deal with measurment error by bigger studies or better measurement? Int. J. Epidemiol. 32, 51–57 (2003). 66. Sullivan, P. F., Eaves, L. J., Kendler, K. S. & Neale, M. C. Genetic case-control association studies in neuropsychiatry. Arch. Gen. Psychiatry. 58, 1015–1024 (2001). 67. Cornelis, M. C., El-Sohemy, A., Kabagambe, E. K. & Campos, H. Coffee, CYP1A2 genotype, and risk of myocardial infarction. JAMA 10, 1135–1141 (2006). 68. Cronbach, L. J. & Meehl, P. E. Construct validity in psychological tests. Psychol. Bull. 52, 281–302 (1955). 69. Hariri, A. R. & Holmes, A. Genetics of emotional regulation: the role of the serotonin transporter in neural function. Trends Cogn. Sci. 10, 182–191 (2006). 70. Leonardo, E. D. & Hen, R. Genetics of affective and anxiety disorders. Annu. Rev. Psychol. 57, 117–137 (2006). 71. Crabbe, J. C. in Behavioral Genetics in the Postgenomic Era (eds Plomin, R., DeFries, J. C., Craig, I. W. & McGuffin, P.) 291–308 (American Psychological Association, Washington, 2003). 72. Flint, J. in Behavioral Genetics in the Postgenomic Era (eds Plomin, R., DeFries, J. C., Craig, I. W. & McGuffin, P.) 425–442 (American Psychological Association, Washington DC, 2003). 73. Cryan, J. & Holmes, A. The ascent of mouse: advances in modelling human depression and anxiety. Nature Rev. Drug Discov. 4, 775–790 (2005). 74. Carola, V., Frazzetto, G. & Gross, C. Identifying interactions between genes and early environment in the mouse. Genes Brain Behav. 5, 189–199 (2006). 75. Holmes, A., Murphy, D. L. & Crawley, J. N. Abnormal behavioral phenotypes of serotonin transporter knockout mice: parallels with human anxiety and depression. Biol. Psychiatry 54, 953–959 (2003). 76. Lipoldova, M. & Demant, P. Genetic susceptibility to infectious disease: lessons from mouse models of leishmaniasis. Nature Rev. Genet. 7, 294–305 (2006). 77. Egan, M. F. et al. Effect of COMT Val108/158Met genotype on frontal lobe function and risk for schizophrenia. Proc. Natl Acad. Sci. 98, 6917–6922 (2001). 590 | JULY 2006 | VOLUME 7 78. Egan, M. F. et al. The BDNF val66met polymorphism affects activity-dependent secretion of BDNF and human memory and hippocampal function. Cell 112, 257–269 (2003). 79. Hariri, A. R., Drabant, E. M. & Weinberger, D. R. Imaging genetics: perspectives from studies of genetically driven variation in serotonin function and corticolimbic affective processing. Biol. Psychiatry 59, 888–897 (2006). 80. Heinz, A. et al. Amygdala-prefrontal coupling depends on a genetic variation of the serotonin transporter. Nature Neurosci. 8, 20–21 (2005). 81. Fallgatter, A. J. et al. Allelic variation of serotonin transporter function modulates the brain electrical response for error processing. Neuropsychopharmacology 29, 1506–1511 (2004). 82. Battaglia, M. et al. Influence of the serotonin transporter promoter gene and shyness on children’s cerebral responses to facial expressions. Arch. Gen. Psychiatry 62, 85–94 (2005). 83. Finley, J. C. et al. A genetic polymorphisn of the α-2adrenergic receptor increases autonomic responses to stress. J. Appl. Physiol. 96, 2231–2239 (2004). 84. Wust, A. F. et al. Common polymorphisms in the glucocorticoid receptor gene are associated with the adrenocorticol responses to psychosocial stress. J. Clin. Endocrinol. Metab. 89, 565–573 (2004). 85. Collins, F. S. The case for a US prospective cohort study of genes and environment. Nature 429, 475–477 (2004). 86. Wright, A. F., Carothers, A. D. & Campbell, H. Geneenvironment interactions — the Biobank UK study. J. Pharmacogenomics 2, 75–82 (2002). 87. Hattori, E., Liu, C., Zhu, H. & Gershon, E. S. Genetic tests of biological systems in affective disorders. Mol. Psychiatry 10, 719–740 (2005). 88. Charney, D. S. Psychobiological mechanisms of resilience and vulnerability: implications for successful adaptation to extreme stress. Am. J. Psychiatry 161, 195–216 (2004). 89. de Kloet, E. R., Joëls, M. & Holsboer, F. Stress and the brain: from adaptation to disease. Nature Rev. Neurosci. 6, 463–475 (2005). 90. Heim, C., Plotsky, P. M. & Nemeroff, C. B. Importance of studying the contributions of early adverse experience to neurobiological findings in depression. Neuropsychopharmacology 29, 641–648 (2004). 91. Hirschhorn, J. N. & Daly, M. J. Genome-wide association studies for common diseases and complex traits. Nature Rev. Genet. 6, 95–108 (2005). 92. Rutter, M., Caspi, A. & Moffitt, T. E. Using sex differences in psychopathology to study causal mechanisms: unifying issues and research strategies. J. Child. Psychol. Psychiatry 44, 1092–1115 (2003). 93. Arseneault, L., Cannon, M., Witton, J. & Murray, R. M. Causal association between cannabis and psychosis: examination of the evidence. Br. J. Psychiatry 184, 110–117 (2004). 94. Henquet, C. et al. Prospective cohort study of cannabis use, predisposition for psychosis and psychotic symptoms in young people. Br. Med. J. 330, 11–15 (2005). 95. Solowij, N. S. et al. Cognitive functioning of long-term heavy cannabis users seeking treatment. JAMA 287, 1123–1131 (2002). 96. Bunney, W. W. & Bunney, B. G. Evidence for a compromised dorsolateral prefrontal cortical parallel circuit in schizophrenia. Brain Res. Rev. 31, 138–146 (2000). 97. Weinberger, D. R. et al. Prefontal neurons and the genetics of schizophrenia. Biol. Psychiatry 50, 825–844 (2001). 98. Henquet, C. et al. An experimental study of COMT Val158-Met moderation of cannabis-induced effects on psychosis and cognition (manuscript in preparation). 99. Castle, D. & Murray, R. Marijuana and Madness (Cambridge University Press, Cambridge, 2004). 100. Hutchison, K. E., McGeary, J., Smolen, A., Brayn, A. & Swift, R. M. The DRD4 VNTR polymorphism moderates craving after alcohol consumption. Health Psychol. 21, 139–149 (2002). 101. Hutchison, K. E. et al. Olanzapine reduces craving for alcohol: a DRD4 VNTR polymorphism by pharmacotherapy interaction. Neuropsychopharmacology 28, 1882–1888 (2003). 102. Hutchison, K. E. et al. The effect of olanzapine on craving and alcohol consumption. Neuropsychopharmacology 31,1310–1317 (2006). 103. Hutchison, K. E., La Chance, H., Naiura, R., Bryan, A. & Smolen, A. The DRD4 VNTR polymorphism influences reactivity to smoking cues. J. Abnorm. Psychol. 111, 134–143 (2002). 104. Slutske, W. S., Caspi, A., Moffitt, T. E. & Poulton, R. Personality and problem gambling: a prospective study of a birth cohort of young adults. Arch. Gen. Psychiatry. 69, 769–775 (2005). 105. Barr, C. S. et al. Rearing condition and rh5-HTTLPR interact to influence limbic-hypothalamic-pituitaryadrenal axis response to stress in infant macaques. Biol. Psychiatry 55, 733–738 (2004). Acknowledgements Supported by the UK Medical Research Council, the National Institute of Mental Health, National Institutes of Health, the William T. Grant Foundation, and Royal Society Wolfson Merit Awards to T.E.M. and A.C. We thank the reviewers for their helpful comments. Competing interests statement The authors declare no competing financial interests. DATABASES The following terms in this article are linked online to: Entrez Gene: http://www.ncbi.nlm.nih.gov/entrez/query. fcgi?db=gene 5-HTT | COMT | DRD4 | MAOA OMIM: http://www.ncbi.nlm.nih.gov/entrez/query. fcgi?db=OMIM ADHD FURTHER INFORMATION Center for Disease Control, Office of Genomics and Disease Prevention: http://www.cdc.gov/genomics Kyoto Encyclopedia of Genes and Genomes (KEGG): http://www.genome.ad.jp/kegg/ UK Biobank: http://www.ukbiobank.ac.uk/ US National Institute of Environmental Health Sciences Environmental Genome Project: http://egp.gs.washington.edu Access to this links box is available online. ONLINE CORRESPONDENCE Nature Reviews Neuroscience publishes items of correspondence online. Such contributions are published at the discretion of the Editors and can be subject to peer review. Correspondence should be no longer than 500 words with up to 15 references and should represent a scholarly attempt to comment on a specific Review or Perspective article that has been published in the journal. To view correspondence, please go to our homepage at: http://www.nature. com/reviews/neuro and follow the link from the Perspective article by Steven Laureys. The following correspondence has recently been published: Confusion about brain death Ari R. Joffe This correspondence relates to the article: Death, unconsciousness and the brain Steven Laureys Nature Rev. Neuroscience 6, 899–909 (2005) www.nature.com/reviews/neuro Too Small to Fail – The New York Times http://www.nytimes.com/2016/06/02/opinion/building-childrens… http://nyti.ms/1RPtnMJ The Opinion Pages | OP-ED COLUMNIST Too Small to Fail Nicholas Kristof JUNE 2, 2016 First, a quiz: What’s the most common “vegetable” eaten by American toddlers? Answer: The French fry. The same study that unearthed that nutritional tragedy also found that on any given day, almost half of American toddlers drink soda or similar drinks, possibly putting the children on a trajectory toward obesity or diabetes. But for many kids, the problems start even earlier. In West Virginia, one study found, almost one-fifth of children are born with alcohol or drugs in their system. Many thus face an uphill struggle from the day they are born. Bear all this in mind as Hillary Clinton, Bernie Sanders and Donald Trump battle over taxes, minimum wages and whether to make tuition free at public universities. Those are legitimate debates, but the biggest obstacles and greatest inequality often have roots early in life: If we want to get more kids in universities, we should invest in preschools. Actually, preschool may be a bit late. Brain research in the last dozen years underscores that the time of life that may shape adult outcomes the most is pregnancy through age 2 or 3. “The road to college attainment, higher wages and social mobility in the United States starts at birth,” notes James Heckman, a Nobel-winning economist at the 1 of 4 8/26/16 5:01 PM Too Small to Fail – The New York Times http://www.nytimes.com/2016/06/02/opinion/building-childrens… University of Chicago. “The greatest barrier to college education is not high tuitions or the risk of student debt; it’s in the skills children have when they first enter kindergarten.” Heckman is not a touchy-feely bleeding heart. He’s a math wiz renowned for his work on econometrics. But he is focusing his work on early education for disadvantaged children because he sees that as perhaps the highest-return public investment in the world today. He measures the economic savings from investments in early childhood — because less money is spent later on juvenile courts, prisons, health care and welfare — and calculates that early-education programs for needy kids pay for themselves several times over. One of the paradoxes of American politics is that this is an issue backed by overwhelming evidence, enjoying bipartisan support, yet Washington is stalled on it. Gallup finds that Americans by more than two to one favor universal pre-K, and Clinton and Sanders are both strong advocates. Trump has made approving comments as well (although online searches of both “Trump” and “preschool” mostly turn up comparisons of him to a preschooler). To be clear, what’s needed is not just education but also help for families beginning in pregnancy, to reduce the risk that children will be born with addictions and to increase the prospect that they will be raised with lots of play and conversation. (By age 4, a child of professionals has heard 30 million more words than a child on welfare.) The best metric of child poverty may have to do not with income but with how often a child is spoken and read to. So it’s in early childhood that the roots of inequality lie. A book from the Russell Sage Foundation, “Too Many Children Left Behind,” notes that 60 to 70 percent of the achievement gap between rich and poor kids is already evident by kindergarten. The book recommends investing in early childhood, for that’s when programs often have the most impact. 2 of 4 8/26/16 5:01 PM Too Small to Fail – The New York Times http://www.nytimes.com/2016/06/02/opinion/building-childrens… It is true that cognitive gains from preschool seem to fade by the third grade, but there are differences in life outcomes that persist. Many years later, these former pre-K students are less likely to be arrested, to drop out of high school, to be on welfare and to be jobless. A wave of recent research in neuroscience explains why early childhood is so critical: That’s when the brain is developing most quickly. Children growing up in poverty face high levels of the stress hormone cortisol, which changes the architecture of the brain, compromising areas like the amygdala and hippocampus. A new collection of essays from Harvard Education Press, “The Leading Edge of Early Childhood Education,” says that this “toxic stress” from poverty impairs brain circuits responsible for impulse control, working memory, emotional regulation, error processing and healthy metabolic functioning. Early-childhood programs protect those young brains. So in this presidential campaign, let’s move beyond the debates about free tuition and minimum wages to push something that might matter even more: earlychildhood programs for needy kids. “It is in the first 1,000 days of life that the stage is set for fulfilling individual potential,” writes Roger Thurow in his powerful and important new book on leveraging early childhood, “The First 1,000 Days.” “If we want to shape the future, to truly improve the world, we have 1,000 days to do it, mother by mother, child by child.” America’s education wars resemble World War I, with each side entrenched and exhausted but no one making much progress. So let’s transcend the stalemate and focus on investing in America’s neediest kids. We rescued banks because they were too big to fail. Now let’s help children who are too small to fail. I invite you to sign up for my free, twice-weekly newsletter. When you do, you’ll receive an email about my columns as they’re published and other occasional commentary. Sign up here. 3 of 4 8/26/16 5:01 PM Too Small to Fail – The New York Times http://www.nytimes.com/2016/06/02/opinion/building-childrens… I also invite you to visit my blog, On the Ground. Please also join me on Facebook and Google+, watch my YouTube videos and follow me on Twitter (@NickKristof). Follow The New York Times Opinion section on Facebook and Twitter, and sign up for the Opinion Today newsletter. A version of this op-ed appears in print on June 2, 2016, on page A23 of the New York edition with the headline: Building Children’s Brains. © 2016 The New York Times Company 4 of 4 8/26/16 5:01 PM